New technology in the analytical cell sciences: the laser scanning cytometer
Louise Reeve and David A. Rew
University Surgical Unit, The Surgical Oncology Research Unit and Laser Cytometry Unit, University of Leicester, Leicester, UK
New technologies are making a major contribution to progress in applied clinical research in surgical oncology. The laser scanning cytometer is a new machine which combines the analytical capabilities of flow cytometry with the ability to inspect and visualize labelled cells and particles. This substantially reduces the uncertainty associated with assays in a wide range of surgical oncology research applications. This article introduces this new technology.
Key words: flow cytometry; laser scanning cytometry.
Introduction
The study of human tissues and tumours is the study of complexity. These biological systems comprise heterogeneous mixtures of cells of varying form, structure, function and viability. Cancer research has been severely handicapped in the past by our limited ability to undertake accurate, quantitative measurement of cellular and subcellular factors in complex samples containing large numbers of cells, in order to improve prognostication and therapy.
Technological innovation drives cancer research. Established technologies in clinical pathology, including conventional microscope-based histopathology and histochemistry, fluorescence microscopy, flow cytometry and computer-based image cytometry, all have limitations. Advanced cellular and molecular biological tools, such as confocal and electron microscopy, while allowing individual cells to be studied in exquisite detail, do not have the speed and versatility to address the key problem of heterogeneity in large cell samples.
A new technology, the laser scanning cytometer (LSC) offers significant advantages in the study of complexity in surgical oncology. In this article, we introduce the technology and place it in the context of existing cell analysis methodologies, with examples of its particular applications in surgical oncology.
Microscope-based techniques for cell analysis
The conventionally stained histological section contains an immense amount of information about tissue architecture, constitution, cell size and characteristics. Histochemical staining aids the identification and the discrimination of markers of interest and allows a degree of quantitation, either by the human observer or by automated image analysis.
The trained human eye and the brain of the cytologist and histopathologist remain the fastest, most efficient and versatile image processor. The human observer possesses the advantages of a high image scan and data acquisition rate, high discriminatory powers, and high interpretative powers. These functions will not be supplanted in the foreseeable future by the computer.
Automated image cytometry harnesses direct visualization and digital manipulation of images of selected cells. It has not proved generally useful to cytopathologists. Nevertheless, human observers are limited to observations in the visual wavelengths, by poor powers of quantitation and by observer fatigue. Data output is constrained to word, writing or artwork. These are areas in which technology and computing can aid the researcher generously.
Fluorescence and laser excitation techniques in cell analysis
Fluorescence-based techniques offer considerable advantages in biological research. Fluorescence is the absorption of light energy (ultraviolet (UV), visible or infra-red (IR)) at one wavelength and its emission at longer wavelengths. The
Educational Section
Stokes shift denotes the difference between the excitation and emission wavelengths, Eₐ/Eₑ. A wide range of organic compounds, including natural and synthetic dyes and drugs, demonstrate fluorescence.² Many can be used to identify biological processes and moieties either directly or when bound to monoclonal antibodies.
Fluorescence excitation can be achieved over a wide range of wavelengths. This is achieved either by using an arc lamp containing a metal vapour such as mercury. Such lamps emit light over a range of constant frequencies. The emissions must be optically filtered to control bandwidth. The alternative is to use a laser, examples of which cover the UV, visible and IR spectral bands. Lasers produce intense monochromatic light in a narrow wavelength, thus giving great specificity of excitation.
Emitted fluorescent light can be visualized directly in a dark field microscope, or collected through optical filters and quantified using photo-multiplier tubes (PMTs), which are also wavelength-specific. These allow for precise and rapid quantification of the emitted light, and thus of the biological moieties attached to the fluorescent markers.
Fluorescence techniques include conventional fluorescence microscopy and confocal microscopy for subcellular analysis. Confocal microscopy is a powerful tool for the study of the three-dimensional infrastructure of individual cells utilizing coherent light. It offers orders of magnitude greater precision in the study of single cells, but has no utility in the quantitative study of large numbers of cells in complex populations such as human tissue samples. Flow cytometry and laser scanning cytometry allow the study of large numbers of cells in biological samples with coarser resolution than is possible with confocal techniques.
Constraints of Fluorescence Technology
All fluorescence-based research is constrained by the physico-chemical characteristics of fluorochromes. Fluorochromes can be used to target most molecular species, either by direct binding (for example, propidium iodide to DNA) or through monoclonal antibody binding. The light absorption and emission profiles generally occupy a broad band of the light spectrum (typically >30 nm), thus limiting the number of fluorochromes in any one experiment, usually to three or less. By increasing the spectral range and number of lasers (for example in the UV or IR bands), or by using polychromatic sources such as mercury arc lamps, additional markers can be studied. Nevertheless, a detailed understanding of fluorescence² is the key to good experimental design.
Flow Cytometry
Flow cytometry (FCM) is a mature technology³ and is the forerunner of the LSC. It has been in widespread use for two decades and will be known to many oncologists. It has been widely used in cancer research for DNA ploidy measurements, proliferation measurements, oncoprotein assays, and the evaluation of drug resistance. Sophisticated applications include cell sorting, rare event analysis, and chromosome sorting as part of the Human Genome Project.
The principle of flow cytometry is to stream particles (cells, nuclei or chromosomes) in suspension through one or more beams of coherent light of defined excitation wavelength. The light source is usually a laser. The laser optics produce a large depth of field and the spot size approximates to that of cells, unlike the much finer beam and resolution found in confocal microscopy.
Particles in the interrogating laser beam scatter light according to their size and granularity. These features give indirect indications of the cell type. Fluorescence emissions can also be studied. Each particle will fluoresce at emission wavelengths according to the physico-chemical characteristics of the fluorochrome dye or dyes used to mark the feature of interest. Up to six parameters of light scatter and fluorescence can be analysed. Large numbers of particles, typically 5000–10,000, can be studied at data collection rates of up to 300 particles per second. Computer processing of list file data facilitates further information analysis and presentation. Information output is in the form of histograms and dot plots, which may be multi-dimensional.
Limitations of Flow Cytometry
A number of practical constraints limit the utility of flow cytometry. One is the requirement to disaggregate tissues into single cell suspensions for analysis by mechanical or enzymatic means. Cells can be damaged in the disaggregation process. The interpretation of FCM data also requires considerable expertise and can be confounded by artefact.
The characterization of cells is indirect, by a combination of cell size and light scattering features. This is acceptable in haematological studies, where leukocytes have precise and reproducible physical characteristics, but is much less useful in the study of cells derived from tissues and tumours. Once past the laser beam, cells cannot be recalled, visualized and reanalysed individually, although subpopulations can be collected using cell sorters which deflect cells by electrostatic charging according to their optical properties. Nevertheless, internal, cell-by-cell verification is not practical using flow cytometry.
Laser Scanning Cytometry
The laser scanning cytometer (LSC)⁴ has evolved from and shares many of the analytical features and components of flow cytometry. The key difference is that in the LSC the laser beam is scanned over the surface of the sample on a constrained surface, the microscope slide, whereas in FCM the particles move past the beam. The laser beam is scanned over the sample on the microscope slide in one axis (y), while the stage is moved in precisely controlled steps by the computer controlled motor in the x-axis. Each cell image thus comprises a large number of pixels (data points) with a 0.5-micron resolution.
The LSC makes some 100 measurements on each cell at cell densities of 100–1000 cells/mm², using a two-dimensional array of values rather than the pulse sort analysis of flow cytometry. Measurements within each array include cell position on the slide, signal area, signal perimeter, peak
Light Excitation Using the LSC
Fig. 1. Diagram of the optical arrangement of a laser scanning cytometer. The instrument can accommodate two lasers and four photomultiplier tubes. The optical output in this particular diagram is linked to a Kontron KS 100 image analyser for further study of colour images of cells under analysis.
fluorescence, time of measurement and texture. Data are initially collected and displayed in the conventional graphical forms used in flow cytometry.
A single cell can be scanned numerous times by the laser beam, with more uniform illumination than is possible with FCM. The speed of scanning of the LSC is such that 1000 cells/min can be analysed. This allows large data sets such as are generated in the study of human tumours and tissues to be collected and analysed in a realistic timeframe.
Light Excitation Using the LSC
The LSC comprises one or two lasers, directional optics to steer the laser beam to the stage of a conventional microscope, light collection optics and up to four photomultiplier tubes to record fluorescence emission and scatter signals from the sample. A Pentium computer with dedicated software controls the LSC and provides a standard interface for data collection and analysis.
The LSC allows light excitation of the sample in a number of ways. Laser excitation derives from a 488 nm blue argon laser, with the option of a second orange or red Helium-Neon laser tuned to either 546 nm or 633 nm. The quoted range of sensitivity of detection for commonly used fluorochromes is as follows. For fluorescein isothiocyanate (FITC) it is 290 - 675 molecules per cell; for phycoerythrin (PE) it is 175 - 360 molecules per cell; and for RPE-CY5 it is 175 - 375 molecules per cell. The coefficient of variation (CV) of the precision of re-measurement is less than 1.0%, and the CV of the accuracy of relocation is 2 - 3%.
The optical system is a conventional microscope. The stage contains a computer-controlled stepper motor which allows precise calculation and recall of each cell position on the sample slide. A CCD camera attached to the microscope allows digitization, display and recording of images (Figs 1 to 3). The microscope can be used in conventional or dark-field modes, thus providing a powerful tool for conventional cytopathology.
An epifluorescence system with a mercury light source is a further enhancement. This allows sample interrogation at a range of other excitation wavelengths, controlled by filters. The machine can thus be considered either as a microscope-enhanced flow cytometer, or as a laser-enhanced fluorescence
Fig. 2. Photograph of the laser scanning cytometer and associated equipment. The body of the instrument contains the laser, control electronics, and the PMTs. The laser beam is steered to the Olympus microscope, to which is also attached the epifluorescence system. The small monitor allows the operator to observe the laser scanning image during the experiment. The prime computer and monitor sit separately. The Image Analyser is linked to the microscope optics by a colour CCD camera.
Fig. 3. This illustrates the optical assembly. Directly above the microscope is a (UVC) colour CCD camera which feeds to the image analysis system. Behind is a smaller black and white camera to aid localization of cells during the operation of the instrument. The epifluorescence system with mercury arc lamp illumination sits below the binocular lenses. Below the microscope stage is the laser reflector and the scatter detector.
Data acquisition and the analysis of cell properties
The laser scanning cytometer provides a range of capabilities of considerable utility in oncology research. The use of the microscope slide offers significant advantages in sample analysis. The machine is optimized for analysis of discrete cells, and sample preparation and disaggregation remains important. Whole cells, intact membranes and cell physiology (for example, cytotoxic drug influx and efflux) are more easily studied.
Because the position (x, y co-ordinates) of the cell or particle on the microscope slide is a measurement property of the machine, the LSC allows recall, visual or camera inspection of specific cells, and the re-evaluation and repositioning of cells after further staining. Individual cells and rare events can be identified within complex cell populations, visualized and analysed directly. This allows confirmation of identity, a critical advance over flow cytometry.
So long as the cells are fixed in position on the slide, they can be restained and remeasured. Multiple studies can be performed on each sample and the data sets combined. Simultaneous analyses can be undertaken with multiple fluorochromes, and the sample can be remeasured to generate time-dependent data, for example drug efflux kinetics.
The microscope slide allows a broad range of sample types to be studied. Small samples in particular can be studied. These include fine needle aspirates, swab and brushings cytology, smear cytology, viscid and mucoid samples such as sputum, imprint specimens (such as from the cut surface of a carcinoma). Many of these analyses are impractical by FCM, because of the sample size or viscosity, for example. Sample suspensions prepared for flow cytometry can also be studied.
Fig. 4. A composite illustration of an oncological application of the LSC in the study of doxorubicin uptake into a human breast tumour cell line (MCF7). (a), (b) and (c) resemble the data output of conventional flow cytometry. By gating on cell size and drug content (a), a subpopulation can be analysed for total (b) and peak (c) drug uptake. This population can be recalled for direct inspection by light microscopy or for further analysis of the digitized image (d) using computer algorithms.
Specific applications
The LSC provides a very broad platform for research. The applications currently foreseen for the LSC may be categorized as those which derive from flow cytometry, e.g. DNA ploidy-- and S-phase fraction analysis, oncoprotein expression and tumour immunophenotyping; and new applications, e.g. drug targeting assays, FISH analysis (vide infra), and new sample presentations, such as smears and fine needle aspirates. It will also be possible to re-evaluate flow cytometric studies with direct visualization to reassess subpopulations and artefact.
Examples of applications for the LSC in surgical oncology
Cytotoxic drug targeting assays
The adoption of a new technology is ultimately governed by the applications for which it can be used. They include the harnessing of the fluorescence characteristics of anticancer drugs to patient-specific assays of cancer chemotherapy. Early studies indicate that the LSC may have particular utility in the development of such assays, which are explained here.
The decision to use adjuvant chemotherapy for primary, metastatic or recurrent tumours, and the selection of particular cytotoxic agents, thereafter, is much influenced by empiricism and individual practice in clinical oncology. This may be depriving many patients with cancers of real benefits from individually targeted adjuvant chemotherapy. The absence of simple clinical assays of cytotoxic drug uptake by individual tumour cells has been a serious handicap. LSC technology allows us to investigate the optimum deployment of existing cytotoxic drugs.
The anthracycline/anthraquinone families of cytotoxic agents, include Adriamycin, daunorubicin, mitoxantrone, epirubicin and idarubicin. These agents have a number of potent cytotoxic actions, which include intercalation of DNA and interference with the topoisomerase proteins (DNA repair enzymes) within the nucleus. They have been widely but sporadically used in the treatment of cancer. Their systemic toxicity has restricted their clinical use. One way to circumvent this problem may be to study means by which their target cell toxicity may be promoted at lower systemic doses. Another may be to identify those tumours which fail to concentrate the agents, and to spare these patients from inappropriate therapy. A third way may be to identify from a panel of agents the drug most reliably concentrated in tumour cells. The anthracyclines are expelled from the cell by the p170 glycoprotein pump which confers multidrug resistance (MDR), and this mechanism may often limit their cytotoxicity.
They also possess the important property of intrinsic fluorescence. They absorb light in the blue region of the visible spectrum (around 470 nm) and emit nearer the red end of the spectrum (around 560 nm). This property has been used as the basis of drug uptake and drug resistance in model systems and malignant ascites. Unfortunately, when applied to disaggregated fresh solid tumour biopsies, such drug assays are confounded by cell heterogeneity of size, origin and viability which severely handicap interpretation (Reeve L and Rew DA, unpublished data).
In vitro studies of tumour cell lines using the LSC (Fig. 4) have indicated that cells from sensitive tumours will exhibit ten-fold or greater drug fluorescence than do resistant cells reversible by p170 inhibitors such as verapamil. Evidence of specific drug uptake or exclusion using the LSC is a considerable advance over the current empiricism inherent in conventional chemotherapy. Incremental clinical benefits may yet be attainable from more scientific use of the existing family of fluorochromatic cytotoxics over a wide range of tumour types.
Fluorescence in situ hybridization (FISH)
The LSC also finds applications in the study of fluorescence in situ hybridization (FISH) markers. FISH is a tool for the identification and measurement of the number of copies of a chromosome or chromosome fragment within an individual cell using gene- and chromosome-specific fluorochrome tagged markers. It can be used for example, to count the chromosome multiples in aneuploid cells. Using the LSC, fluorescent chromosome-specific markers can be identified and quantified with coarse resolution. Thus, for example, for the first time we are able to quantify chromosomal aneuploidy in complex cell samples from tumours.
Concluding comments
Laser scanning cytometry is a significant new technology for research in the analytical cell sciences within the remit of surgical oncology and many other disciplines. It offers considerable advantages over earlier technologies. There remain a number of issues to be resolved before the full range of capabilities and limitations of laser scanning cytometry are understood, and we may expect further technical innovation, as has been the case since flow cytometry was introduced 20 years ago. The technology should allow a more rigorous evaluation of laser fluorimetric data, and of cell and tissue heterogeneity. The challenge for surgical oncologists and for laboratory scientists is to exploit its considerable potential for practical assays with which to use it to improve diagnosis, prognostication and therapy.
References
Rew DA. Heterogeneity, biodiversity and bioperversity in human solid tumours. Eur J Surg Oncol 1996; 22: 469-73.
Haugland RP, et al. Handbook of Fluorescent Probes and Research Chemicals (6th edn). Eugene, Oregon: Molecular Probes Inc.; 1996.
Watson JV. An Introduction to Flow Cytometry. Cambridge: Cambridge University Press; 1992.
Luther E, Kamentsky LA. Resolution of mitotic cells using laser scanning cytometry. Cytometry 1996; 23: 272-8.
Kamentsky LA, Kamentsky LD. Microscope based multimparameter laser scanning cytometer yielding data comparable to flow cytometry data. Cytometry 1991; 12: 381-7.
Martin-Reay DG, Kamentsky LA, Weinberg DS, Hollister KA, Cibas ES. Evaluation of a new slide based laser scanning Educational section
cytometer for DNA Analysis of tumours. Anat Pathol 1994; 102: 432-8.
Sasaki K, Kurose A, Miura Y, Sato T, Ikeda E. DNA ploidy analysis by laser scanning cytometry in colorectal cancers, and comparison with flow cytometry. Cytometry 1996: 23: 106 9.
Clinch RJ, Wallach JL, Zutter MM, Kamentsky LA. Immunophenotypic analysis of haematologic malignancy by laser scanning cytometry. Am J Clin Pathol 1997; (in press).
Kamentsky LA, Kamentsky LD, Fletcher JA, Kurose A, Sasaki K. Methods for automatic multiparameter analysis of fluorescence in situ hybridised specimens with a laser scanning cytometer. Cytometry 1997: 27: 117-25.
Krishnan A, Ganapathi K. Laser flow studies on the intracellular fluorescence of anthracyclines. Cancer Res 1980; 40: 3959-900.
Krishan A, Sheldrick KS, Davila E, Vogel C, Sternheim W. Patterns of anthracycline retention in human tumour cells. Cytometry 1987; 8: 306-14.
Herweijer H, Van den Engh G, Noortek K. A rapid and sensitive flow cytometric method for the detection of multi-drug resistant cells. Cytometry 1989; 10: 463-8.
Gheuens EE, van Bockstaele DR, van der Keur M, Tanke HJ, van Doomert AT, De Bruijn EA. Flow cytometric double labelling technique for screening of multidrug resistance. Cytometry 1991; 12: 636-44.
Kristian A, Sauterj C. Flow cytometric monitoring of cellular resistance to cancer chemotherapy. In: Bauer KD, Duque RE, Shankey TV (eds) Flow Cytometry: Principles and Clinical Application. New York: Williams and Wilkins. 1992: 459-67.
Smith JR, Sykes HR, Fox ME, Furlong IJ. Subcellular distribution of mitoxantrone in human and drug resistant murine cells analysed by flow cytometry and confocal microscopy. Cancer Res 1992; 52: 4000-8.
Carpenter T, Goslrees MC, Desoize B. Evaluation of a method for the detection of cells with reduced drug retention in solid tumours. Cytometry 1992; 13: 630-7.
Self-assessment exercise
Based on the Educational Section in the August 1997 issue of The Journal.
"Current concepts about testicular cancer" by J. P. van Basten, H. Schraffordt Koops, D. Th. Sleijfer, E. Pras, M. F. van Driel and H. J. Hoekstra and "The management of patients with advanced cancer (III), by S. D. Heys, D. Currie and O. Eremin.
(Answers given on p. 454)
Modern treatment (cisplatin-based combination chemotherapy, surgery and radiotherapy) can achieve up to 90% long-term survival with acceptable morbidity in young males presenting with testicular cancer.
The incidence of testicular cancer is highest in young Caucasian males; a four-fold incidence has been reported over the past four decades.
Of the many factors postulated in the aetiology of testicular cancer only the following are believed to play a key role—undescended testes, previous history of testicular cancer and infection with HIV.
Do tumour markers play an important role in establishing the diagnosis, prognosis and the likely outcome of treatment.
Cisplatin-based chemotherapy has substantially altered the management of patients with testicular cancer.
Bilateral retroperitoneal lymph node dissection is crucial for both early and advanced disease.
The morbidity of cisplatin-based combination chemotherapy is minimal as is that of retroperitoneal node dissection.
Pain is a common feature in patients with cancer. Medical management of such patients should follow established pharmacological protocols.
Surgical procedures have no role to play in pain control in patients with cancer.
The spinal column is a common site of metastatic spread, involving vertebrae and/or spinal canal (extradural space).
Spinal cord compression may occur and is usually readily diagnosed.
The standard investigation for bone metastases is an isotope bone scan and for spinal cord compression it is a myelogram.
Spinal cord compression is dealt with as an urgent but not acute oncological problem.
Intracranial metastases are a rare occurrence with tumours. There is little scope for successful surgical intervention in such patients.
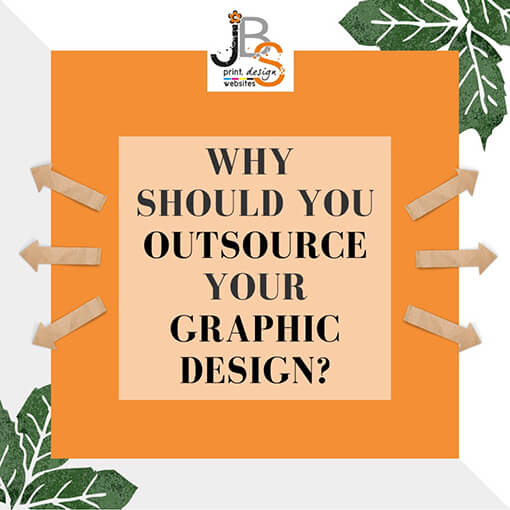
Why do we use it?
It is a long established fact that a reader will be distracted by the readable content of a page when looking at its layout. The point of using Lorem Ipsum is that it has a more-or-less normal distribution of letters, as opposed to using 'Content here, content here', making it look like readable English. Many desktop publishing packages and web page editors now use Lorem Ipsum as their default model text, and a search for 'lorem ipsum' will uncover many web sites still in their infancy. Various versions have evolved over the years, sometimes by accident, sometimes on purpose (injected humour and the like).